By Joseph C. Benedyk, Editor.
Introduction
For decades, additive manufacturing (AM), also known as 3D printing (3DP), has grown and proliferated worldwide into a multibillion dollar industry encompassing many different technologies and types of materials. AM methods embody a group of emerging technologies that create a functional 3D object from any one of a variety of materials, including aluminum alloys, by adding material a layer at a time until the final shape is achieved.[1]
The AM equipment utilizes computer controlled production methods based on digital data from a specific 3D model or computer aided design (CAD) data. In the case of metal shapes, the differences between AM methods and traditional subtractive or formative methods (extrusion, forging, shape casting, machining, grinding, etc.) bear consideration in lieu of the comparative economics of each of these methods with respect to material and production costs. Compared with traditional methods of producing metal shapes, metal AM has the ability to manufacture shaped objects with almost unlimited geometries directly from powder metal or wire. It also can produce components with a lighter weight, reduced assembly/joining requirements, less metal scrap, and without the need for tooling. On the positive side, metal AM has developed niche markets for prototyping and limited production parts; but on the negative side, the production costs and slow print/deposit speed of AM systems retard its potential for mass production in the near future.
Today, AM applies across the board relative to material types from plastics to metals, even ceramics and composites, and is growing rapidly in production volume and economic impact. The aluminum 3D objects produced today using AM methods in most cases refer to localized and selective fusion bonding utilizing digitally controlled laser or electron beam sources of aluminum alloy powders laid down in layers by stereolithography (3DP). However, other AM methods utilizing aluminum alloy wire are proliferating as the potential of AM is being realized for the manufacture of prototype and limited production parts.
Market analyses show a significant growth pattern for AM manufacturing, extending into the near future (Figure 1).[2] According to the Wohlers Report 2017, there were 97 manufacturers globally that were producing and selling AM systems in 2016 with nearly half of the service providers surveyed running AM systems that produce metal parts.[3]

Progress on AM Standards and Specifications
It was only in 2012 that the American Society for Testing Materials (ASTM) committee on AM technologies (F42) published their ASTM F2792-12a standard, which sorted out AM technologies by process methodology involving the fabrication of 3D objects through deposition and bonding of material. The F42 ASTM committee was formed in 2009 and today has a current membership of over 500, representing 25 nations and with six technical subcommittees that set standards addressing all aspects of AM technologies.
In 2011, ASTM and the International Organization for Standardization (ISO) made an agreement to create joint AM standards, allowing synergy between both organizations’ committees (ASTM F42 and ISO TC261) and establishing a common set of future ISO/ASTM standards on AM. Prior to this merger, the ASTM published five standards on AM manufacturing, while the ISO had published three standards.
Together, the ASTM and the ISO created a new standard in 2015, ISO/ASTM 52900-15, Additive Manufacturing – General Principles – Terminology, which established the following definition for AM: “A process of joining materials to make parts from 3D model data, usually layer upon layer, as opposed to subtractive and formative manufacturing methodologies.”[4] Machining and grinding processes qualify as subtractive methodologies, while extrusion, forging, rolling, casting, etc. qualify as formative methodologies.
The ISO/ASTM 52900-15 standard further divided AM processes into multi-step or single step fabrication methods. It also established seven categories of AM, all of which apply to plastic materials. However, three of these AM processes generally apply to metal fabrication, including aluminum and titanium alloy fabrication in particular, such as:
-
- Directed Energy Deposition (DED) – an AM process in which focused thermal energy (e.g., laser, electron beam, electric arc, or plasma arc) is used to fuse wire or powder materials by melting and solidification (Figure 2)
- Powder Bed Fusion (PBF) – an AM process in which thermal energy selectively fuses regions of a powder bed, most commonly known as selective laser sintering (SLS), selective laser melting (SLM), or electron beam melting (EBM) (Figure 3)
- Sheet Lamination (SL) – an AM process in which sheets of material are bonded to form an object, with ultrasonic welding typically used (UAM) to create solid state bonds between successive layers of metal foils or sheets in tape form, which can be single or multiple types of metallic materials. Computer numerical control (CNC) machining is sometimes done to remove material to create a desired shape (Figure 4) [5]
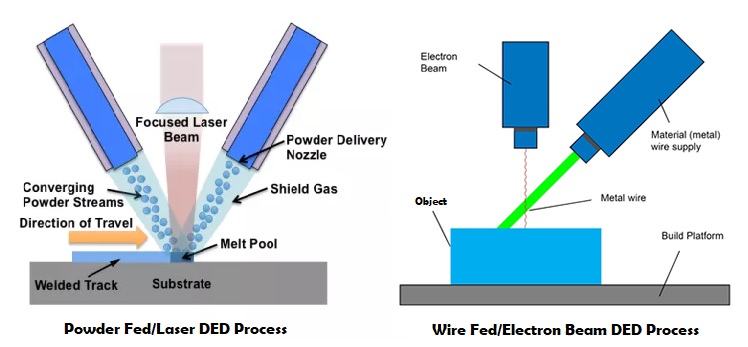

- ISO/ASTM 52921-13 – Standard Terminology for Additive Manufacturing, Coordinate Systems and Test Methodologies
- ISO/ASTM 52915-16 – Standard Specification for Additive Manufacturing File Format (AMF) Version 1.2
- ISO/ASTM 52910-17 – Standard Guidelines for Design for Additive Manufacturing
- ISO/ASTM 52901-17 – Additive Manufacturing-General Principles-Requirements for Purchased AM Parts
At the same time, several working groups within ISO and ASTM are hard at work to establish new AM specifications that apply to specific metallic materials and AM process parameter control and post processing, among others. In addition several technical organizations, such as ASME, AWS, and SAE AMS have since engaged in their own AM standards development.
In February 2017, the Additive Manufacturing Standardization Collaborative (AMSC) consisting of the America Makes organization, (also known as the National Additive Manufacturing Innovation Institute and consisting of members from hundreds of companies and institutions, including the Aluminum Association), together with the American National Standards Institute (ANSI), published a roadmap for standardizing AM.[6] This AM roadmap, identified existing standards and standards in development, focusing on the industrial AM market especially for aerospace, defense, and medical applications. It identifies a total of 89 gaps in published standards or specifications in areas of design, precursor materials, process control, post-processing, and finished material properties, as well as certification, nondestructive evaluation, and maintenance.
Without firm standards on dimensional tolerances and especially mechanical properties of aluminum alloys produced by any of these AM processes, the market has depended for the most part on data from AM companies promoting “typical” characteristics and properties. For the most part, aluminum alloys used for PBF type AM processes are casting alloys, while wrought aluminum alloys are used as wire feedstock in DED type processes and wrought aluminum alloy sheet/foil is used in SL type processes. Consider that the DED and PBF AM methods of producing aluminum alloy components basically involve localized melting and solidification, while the SL method produces aluminum alloy components in the solid state.
The evolving standards scenario described above is common for such a rapidly evolving process technology. In fact, most aluminum alloy AM parts are made by PBF methods, and the powder metallurgy industry has already established many standards and test procedures for evaluating metallic powders and products produced by powder metallurgy methods. The majority of metal AM parts are used for prototypes or in low volume production in the markets served. Testing of the final product for functionality in use is done universally as final proof of product.
At the same time, networking with AM equipment and service providers is the best way to learn about the potential of metal AM for particular applications. Several venues for technical information gathering and collaboration in this respect include professional societies, such as TMS, AWS, ASM International, SAE, etc., which more and more include metal AM presentations in their conferences and meetings.[7]
Aluminum Alloys Used in AM Processes
Aluminum Alloy Powder as AM Feedstock
For PBF or DED AM processes, aluminum alloy powders consist primarily of hypereutectic casting alloy compositions, e.g., typically AlSi10Mg, AlSi12, AlSi12Mg alloys, and F357 of a spherical shape and particular size distribution, typically between 10 to 75 microns. Unlike the irregular powder particles used in powder metallurgy (PM) processing, metal injection molding (MIM) and AM processes require spheroidal or spherical particle shapes to achieve proper flow characteristics in the process and a proper particle size range to achieve good mechanical properties (Figure 5). These metal AM particle characteristics are achieved by controlled atomization under inert gas to maintain oxygen contents below 0.3% and final particle size classification.[8]
It should be noted that, as with all types of aluminum powders, they need to be stored under inert gas in dry and cool conditions to prevent oxidation and moisture pickup, and adequate ventilation and respiratory protection is required when the powder is used for PBF. There is also the danger of aluminum dust explosions in confined spaces, and these powders can react with water and other reagents releasing hydrogen gas, which can ignite spontaneously.
Mechanical Properties of PBF Manufactured Aluminum Alloy Parts
The main process variables in PBF of AlSi10Mg alloy, starting with the shape and powder size distribution to the laser power and speed, layer thickness, and scanning strategy, have a major effect on the mechanical properties of the final parts. Post heat treatment also affects microstructure and properties. As the SLM process introduces high thermal stresses (much as welding does), it is beneficial to reduce these by either using a heated platform in the SLM process or conducting stress relieving treatment on SLM parts made of AlSi10Mg alloy; however, stress relieving reduces the tensile strength of the as-built parts while increasing the ductility.
In studying these variables for selective laser melting or SLM (trade name for a PBF process per ISO/ASTM 52900-15) of AlSi10Mg, it was found that the amount of laser energy input necessary to reduce porosity to the minimum was approximately 60 J/mm3. At the same time, AlSi10Mg parts produced by SLM had a much higher hardness and tensile strength than traditionally cast parts due to the ultrafine grained microstructure resulting from the rapid localized fusion and quenching SLM conditions, although subsequent heat treatment significantly modified the microstructure (Figure 7) and mechanical properties, usually reducing strength and raising ductility.[11-12]

Aluminum Alloy DED Parts from Wire
When comparing aluminum alloy wire feedstock to powder, whether the heat source is laser, electron beam, or wire arc, a wide variety of wrought alloy options exist for wire feedstock. In the electron beam additive manufacturing (EBAM™) process, exclusive to Sciaky, Inc., aluminum alloy wire choices include 1100, 2318, 2319, 3000 series, 4043, 4047, 5183, 5356, 5554, and 5556. This process is conducted inside a vacuum chamber where Sciaky’s electron beam gun deposits metal from wire feedstock layer by layer at deposition rates considered the fastest of all metal AM processes, especially compared with laser powder or wire DED systems, for the manufacture of parts up to many meters in size, limited only by the size of the vacuum chamber.[14]
The other important DED process using wire feedstock is wire plus arc additive manufacturing (WAAM) in which argon shielded welding with wire is robotically controlled to produce parts of almost unlimited lengths. Aluminum wire alloys, for example, used at the Cranfield University WAAM deposition facility, capable of manufacturing parts up to 10 m in length, in the U.K. have included 2024, 2319, 4043, and 5087.[15] This facility is equipped to conduct WAAM processing with dual robots and parallel processing, i.e., deposition with NDT, machining, and/or cold working. Among the aluminum alloy AM parts made there by the WAAM process was a 6 m long spar weighing 300 kg (Figure 8).[16]

Aluminum Alloy SL/UAM Parts from Sheet/Foil
As shown in Figure 4, UAM combines ultrasonic joining of thin metal tapes, in the case of aluminum from any wrought alloy sheet/foil tape, combined with CNC milling to produce near net parts. As with other AM processes, process parameters must be optimized to achieve good results. For SL/UAM, the process parameters of ultrasonic weld force, speed, amplitude, and temperature must be optimized to achieve near 100% density (Figure 9). In the case of annealed 6061 tape (0.006-inches thick) that was used to produce shapes layer-by-layer using a UAM Fabrisonic Sonic Layer 4000TM machine operating at 9 kW, maximum mechanical layer strength was achieved at a weld amplitude of 32.76 microns and weld speed of 84.6 mm/s.[17] The uniqueness of the UAM process allows manufacturers to create novel functionality in terms of embedded circuitry for use in electronics (Figure 10), mixed aluminum/copper composites for heat exchangers, etc.[18]


Economics of Metal AM
Recent Economic Trends
In 2014, the global AM market was $2.6 billion and there was an estimated $667 million in value-added (gross income) produced using AM, which equated to 0.01% of total global manufacturing (U.S. value-added AM for 2014 was estimated at $241 million). The percentage breakdown of AM revenue streams for the AM market at that time, out of a total of $2.6 billion, was 46.5% for systems and services (excluding consumer), 30.3% for on-demand parts, 18.6% for materials, 2.3% for software, and 2.3% for consumer systems and service. For 2014, the metal AM market accounted for about 10% of total global AM business activity.[19-20]
According to the Wohler Associates 2016 report on AM, with the global economy at about $80 trillion and manufacturing accounting for $12.8 trillion or about 16% of the total, global AM at about $5.2 billion represented about 0.04% of all manufacturing in 2015.[21] Out of the $5.2 billion AM market in 2015, the AM materials market amounted to $768.5 million, of which polymers (polymer powder, filaments, and photopolymer) represented about 85% and AM metal materials (powder, wire) represented 11.4% of the total AM materials market, while the remainder included binder jet, wax, and sheet materials. With caution, the sales projection in this report for AM products and services for 2017 was about $8.8 billion worldwide.
Metal AM for Prototyping and Larger Scale Production
The metal AM market has been primarily used as a tool for prototyping or for limited production, making a few shapes at a time, but its potential for volume industrial production was ripening in aerospace, medical/dental, automotive, and energy markets. To assess the business case for AM part production requires consideration of the direct costs of metal AM compared with conventional manufacturing costs for subtractive and formative processes as well as accounting for indirect factors that add value to customers, e.g., lighter weight, manufacturing parts that cannot be made by traditional methods, rapid delivery, etc.
Thomas and Gilbert conducted a review of the extensive literature of AM costs and benefits based on the 2011 timeframe.[22] At that time and even true today, AM parts are considered cost effective in limited production. Some of the data analyzed in their review compared costs of AM aluminum parts produced by the SLS process and high pressure die casting (HPDC), which is instructive as both methods create 3D shapes from aluminum casting alloys (Figure 11). As seen, 42 SLS parts, even with modifications that exploit SLS potential, constitute the breakeven point for this part, taking account of the high mold costs for the HPDC process and the much higher material costs for the aluminum alloy feedstock for the SLS process (surprisingly, a 10:1 ratio).

Even though it was designed for rapid prototyping, AM aims at direct rapid manufacturing of final parts, based on the potential for on-demand and on-location manufacture of customized parts and the geometric freedom it offers. Analysis of service provider quotes of PBF components for 2014 has shown that the cost per cm3 of aluminum SLA and SLM parts is volume sensitive and decreases to a threshold value after about 100 cm3 (Figure 13).[24]

Die Cladding and Manufacture
A substantial amount of work has been done at Case Western Reserve University (CWRU) on the applications of AM for either cladding or building dies and die components for HPDC.[25] As most of these dies are made from H13 steel, similar to that of aluminum extrusion and forging dies, this work has relevance universally. The CWRU analysis indicated that die/tooling rejuvenation can be cost effective when the damaged layer is small (say 0.100 inch or less) and removed by machining; when the recessed layer is rebuilt by AM methods with maraging steel, the rebuilt die/tooling has a higher tensile strength at high temperatures compared with H13 steel. If H13 steel is deposited by a similar process, it forms a hard layer with lower toughness which may be prone to cracking.
Other work on die/tooling repair and manufacture using various DED and even PBF processes with wire or powder forms of hot work tool steels, including H13, H11, CPM 9V, and maraging 250, indicate real possibilities for die makers across the board in the field of formative metalworking processes.[26-28]
Conclusions
Addressing the question as to whether AM of aluminum alloys augments or challenges traditional manufacturing, the answer can be summed up in one word, “Frenemy.” On the positive side, aluminum AM processes open up markets not available to traditional aluminum manufacturers in terms of part complexity or customer requirements for rapid prototyping and delivery. Another positive, some mold makers in the aluminum industry look to hot work steel AM for repair and even die building; in fact, Light Metal Age will publish an article by Prof. Barbara Reggiani and her team at the University of Bologna on hot work steel AM for aluminum extrusion dies in its forthcoming June issue.
However, on the negative side, the subtractive manufacturing segment of the aluminum industry faces the biggest challenge from not only metal but also polymer AM. This is especially true for aluminum parts that are made in limited production quantities, especially when some of the high strength AM polymers can also be substituted and when time to complete and deliver is a driver.
References
- “3D Printing,” Light Metal Age.
- Wile, Rob, “CREDIT SUISSE: 3D Printing Is Going To Be Way Bigger Than What The 3D Printing Companies Are Saying,” Business Insider, September 17, 2013.
- Goehrke, Sarah Anderson, “Wohlers Report 2017: The Real Authority on 3D Printing,” 3DPrint.com, April 5, 2017.
- ISO/ASTM 52900:2015(en) – Additive manufacturing – General principles – Terminology.
- “3D metal printing technology without the compromise,” Fabrisonic.
- Standardization Roadmap for Additive Manufacturing, Version 1.0, Prepared by: America Makes and ANSI Additive Manufacturing Standardization Collaborative (AMSC), February 2017.
- FormNext.
- Joys, J., R. Kasler, and L.R.Thomas, “Selecting Atomized Aluminum Powders for the Metal Additive Manufacturing Process,” Metal Additive Manufacturing, Fall/Autumn, Vol. 2, No. 3, 2016, pp. 71-76.
- Wang, L., Y. Liu, and S. Chang, “Fabrication of Spherical AlSi10Mg Powders by Radio Frequency Plasma Spheroidization,” Metallurgical and Materials Transactions A, Vol. 47A, May 2016, pp. 2,444-2,453.
- Martin, J.H., et al., “Recent Advances in Additive Manufacturing of High Strength 7000 Series Aluminum,” Advanced Materials & Processes, Vol. 176, No. 1, January 2018, pp. 18-22.
- Trevisan, F., et al., “On the Selective Laser Melting (SLM) of the AlSi10Mg Alloy: Process, Microstructure, and Mechanical Properties” Materials, 10 (1), 76, 2017.
- Manfredi, D. et al., “Chapter 1: Additive Manufacturing of Al Alloys and Aluminum Matrix Composites (AMCs),” Light Metal Alloys Applications, INTECH, June 11, 2014, p. 32.
- “Materials Guide,” Sintavia.
- “Advantages of Wire AM vs. Powder AM,” Sciaky Inc.
- Williams, S., “Large Scale Metal Wire + Arc Additive Manufacturing of Structural Engineering Parts – Slide Presentation,” Cranfield University, U.K., July 13, 2016.
- WAAM – Welding Engineering and Laser Processing Centre.
- Wolcott, P.J., A. Hehr, and M.J. Dapino, “Optimized Welding Parameters for Al 6061 Ultrasonic Additive Manufactured Structures,” Journal of Materials Research, Vol. 29, No. 17, September. 14, 2014, pp. 2,055-2,065.
- Friel, R.J. and R.A. Harris, “Ultrasonic Additive Manufacturing – A Hybrid Production Process for Novel Functional Products,” Procedia CIRP, Vol. 6, 2013, pp. 35-40.
- Thomas, D. “Costs, Benefits, and Adoption of Additive Manufacturing: A Supply Chain Perspective,” International Journal of Advanced Manufacturing Technologies, Vol. 85, 2016, pp. 1,857-1,876.
- Hurn, J. and T. Li,, SLM Solutions Group AG (AM3D.DE), Credit Suisse Small and Mid-cap Research Report, June 23, 2014.
- Caffrey, T., T. Wohlers, and R.I. Campbell, “Executive Summary of the Wohlers Report 2016,” Wohlers, Fort Collins, CO, 2016.
- Thomas, D. and S.W. Gilbert, “Cost and Cost Effectiveness of Additive Manufacturing: A Literature Review and Discussion,” NIST Special Publication 1176, December 2014.
- Yoon,H.-S., et al., “A Comparison of Energy Consumption in Bulk Forming, Subtractive, and Additive Processes: Review and Case Study,” International Journal of Precision Engineering and Manufacturing-Green Technology, Vol. 1, No. 3, July 2014, pp. 261-279.
- Baldinger, M., et al., “Additive Manufacturing Cost Estimation for Buy Scenarios,” Rapid Prototyping Journal, Vol. 22, No. 6, 2016, pp. 871-877.
- Schwam, D., “Additive Manufacturing for Die Casting Applications,” paper presented at the NADCA 2015 Die Casting Congress and Exposition.
- Xue, L, J. Chen, and S.-H. Wang, “Freeform Laser Consolidated H13 and CPM 9V Tool Steels,” Metallography, Microstructure, and Analysis, Vol. 2, 2013, pp. 67-78.
- Kottman, M., et al., “Laser Hot Wire Process: A Novel Process for Near-net Shape Fabrication for High Throughput Applications,” Journal of Metals, Vol. 67, No. 3, 2015, pp. 622-628.
- Klocke, F., et al., “State-of-the-art Laser Additive Manufacturing for Hot Work Tool Steels,” Procedia CIRP, Vol. 63, 2017, pp. 58-63.
Editor’s Note: This article first appeared in the February 2018 issue of Light Metal Age. To read more articles from this issue, please subscribe.
Joseph C. Benedyk (Dr. Joe) is a research professor at the Illinois Institute of Technology (IIT) working to establish cooperative industry/ university research programs in the fields of heat treatment, casting, and extrusion of aluminum and aluminum alloys. In 2013, the Aluminum Extruders Council honored Dr. Joe with a plaque in recognition of his volunteer spirit, dedication to the aluminum extrusion industry, and his willingness to selflessly share his knowledge with others. His current work at the IIT thermal processing center involves employing multiple steps in the age hardening process of age hardenable aluminum alloys in order to significantly reduce the overall aging times required to achieve target properties for a given temper. He has contributed over 132 articles to Light Metal Age and serves as editor covering applications and technology of light metals.